During the past 2 decades, PK/PD data and models both for efficacy and for safety have been developed in almost all therapeutic areas, showing a clear benefit in the drug development process. There are areas, however, where PK/PD is still an exception, and one of those areas is gene therapy.
Cardiac therapeutic gene delivery is still reminiscent of a biological blackbox where the input and output are defined between the administered dose of a therapeutic vector and its biological effect, that is, on cardiac contractile performance or remodeling, respectively, whereas the inner workings of the system remain enigmatic. To date, most preclinical studies that reported improvement of experimental HF using cardiac therapeutic gene delivery were limited to a single vector dosage and 1-time estimation of cardiac transgene expression levels, mainly at the study end point. The current lack of understanding the PK/PD underlying therapeutic effects will eventually delay translation and design of proper clinical trials. Systematic acquisition of quantitative PK/PD data during preclinical development is inevitable to assess whether the therapeutic product is reasonably safe for initial testing in humans, and a prerequisite for investigational new drug application to obtain legal drug status.
In the case of PK, referring to “what the body does with the drug,” quantitative characterization of the biotransformation, biodistribution, and excretion would allow for describing and understanding the effects elicited by the gene therapy system. Here, biotransformation is defined as the process during which the therapeutic gene sequence packaged in any vector is converted into a potentially therapeutic gene product in the target cell. Although the vector has to overcome several biological barriers after intravascular or intramyocardial delivery that could be described by conventional PK models, biotransformation of a therapeutic gene product uses the innate cellular transcriptional and translational machinery referring to a new concept of intracellular PK. The process thus integrates (1) efficiency of vector uptake, (2) (in the case of AAV) dynamics of nuclear trafficking and escape from vector degradation, (3) mRNA transcription rate and stability, (4) eventual translation, and (5) degradation of the gene product. For gene therapy to be successful, it is essential to describe the net effect of intracellular biotransformation systematically in terms of its time course and level of cardiac transgene expression. Stable long-term expression seems to be mandatory for the treatment of chronic HF. Defining the kinetics and dose–response relationship among the therapeutic vector system, transgene expression, and therapeutic efficacy is thus necessary to determine whether the particular combination is appropriate and safe.
With cardiac gene transfer where vector delivery to myocardial tissue involves direct physical methods, the potential for the wider distribution of the vector exists, and this may result in untoward effects on distant organs. Available biodistribution and excretion data from preclinical studies in small and large HF animal models have assessed either viral transduction or transgene expression of noncardiac tissues. But they were mostly limited to nonsystematic analysis of a single vector dosage and lacked comprehensive organ and tissue assessment including reproductive organ systems and body fluids. Noninvasive imaging of transgene expression particularly in large animal models approximating human size and weight may change the way transgene expression is defined. Taking advantage of advances in molecular imaging might allow for PET- or SPECT-based serial in vivo imaging of transgene expression and biodistribution alike. A suitable example of this is imaging of either cardiac-targeted thymidine kinase or sodium iodine symporter gene expression with the use of labeled and radioactive substrates used in clinical routine. Application of the clinically established technology in large animal preclinical studies is expected to advance our understanding of the kinetics of expression and biodistribution greatly.
With respect to PD, defined as “what the drug does to the body,” a major concern in the field of gene therapy is the immune response to vector delivery, as well as vector- and transgene-associated toxicity that requires special consideration in clinical studies. Viral vectors such as Ads have the potential of inducing significant immunologic responses that limit transgene expression and may cause collateral tissue damage or autoimmune response. Hence, defining the mechanisms of immunologic responses and the maximally tolerated dose for each vector and delivery route should be a priority in preclinical development. AAV vectors have attracted the interest of gene therapists worldwide because of several features, but chief among these is the virus apparent lack of pathogenicity. Further development of wild-type AAV as therapeutic vectors has eliminated its ability to integrate into the host genome at a specific site (designated AAVS1) in the human chromosome 19 by removal of the rep and cap from the DNA of the vector.
Innate immune response to AAV vectors occurs in the form of transient increase of inflammatory cytokines and some liver infiltration of neutrophils, which together with macrophages seem to sequester a large percentage of circulating AAV particles. Given the rather modest innate response, AAV instigates robust humoral immunity both in animal models and in humans, where 80% to 90% of individuals are thought to be seropositive for AAV2 antibodies. Of those, ≈30% showed neutralizing ability and they can drastically mitigate vector transduction efficiency. Importantly, the humoral immune response to 1 serotype can give rise to a complex profile of serum antibodies capable of binding and neutralizing various AAV serotypes. In addition to persistent AAV-specific antibody levels, it seems from prime-boost studies in animals and from clinical trials that the B-cell memory is also strong. In a clinical trial using an AAV2-based vector to treat hemophilia B, targeted destruction of transduced liver cells resulted from a T-cell–mediated response to this AAV serotype. Apparently, the T lymphocyte response against AAV2 is susceptible to prednisolone and it is currently unclear whether the cytotoxic response equally extends to all serotypes. Of similar interest is the recent observation that the humoral response to AAVs seems to be T-cell dependent; anti-CD4 antibodies inhibiting T-cell function prevented antibody formation and allowed vector readministration. Notably, only 1 patient in the CUPID trial that uses AAV1 showed transient positivity in an enzyme linked immunospot assay indicating a cellular immune response against the AAV1 capsid.
Because AAVs are currently among the most frequently used viral vectors in clinical trials, and still little is known about variability of AAV-induced immunity, clinical trials should make every attempt to pool available data and enable a deeper understanding of AAV–host interactions for the safe and efficient use of AAV as a gene transfer vector. Although in its infancy, unraveling PK/PD data for gene-based therapeutics is expected to provide a clear benefit in the gene-based drug development process and safety for clinical use.
Source ahajournals.org
DUC TIN Surgical Clinic
Tin tức liên quan
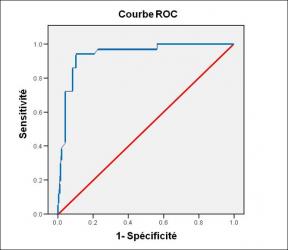
Performance diagnostique de l’interféron gamma dans l’identification de l’origine tuberculeuse des pleurésies exsudatives
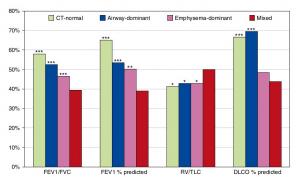
A Mixed Phenotype of Airway Wall Thickening and Emphysema Is Associated with Dyspnea and Hospitalization for Chronic Obstructive Pulmonary Disease.
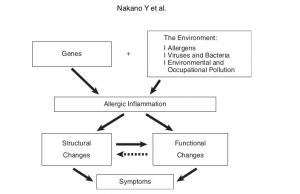
Radiological Approach to Asthma and COPD-The Role of Computed Tomography.
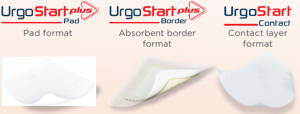
Significant annual cost savings found with UrgoStart in UK and Germany
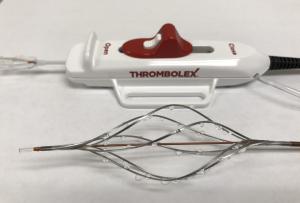
Thrombolex announces 510(k) clearance of Bashir catheter systems for thromboembolic disorders
Phone: (028) 3981 2678
Mobile: 0903 839 878 - 0909 384 389